INTRODUCTION
In recent years, wearable hip exoskeletons, designed to enhance gait function and quality of life, have been em-ployed to improve walking ability, activities of daily living, and social interaction in elderly individuals and those with gait impairments.1,2 Rehabilitation using wearable hip exoskeletons provides repetitive and high-intensity training, and their accessibility allows continued rehabilitation without the need for visits to medical facilities.2 These devices not only assist users in performing daily activities independently, but also facilitate frequent rehabilitation training, leading to more effective outcomes.2 In addition, wearable hip exoskeletons have been shown to improve gait in elderly individuals and patients with gait impairments by reducing muscle effort and energy consumption.1
A wearable hip exoskeleton provides assistance and re-sistance by applying torque to the flexion and extension of the hip joint.3 Previous studies have reported that walking with a wearable hip exoskeleton, compared to walking without one, significantly improves walking speed, respiration, and metabolic energy expenditure.3–5 The assistive torque of the wearable hip exoskeleton enhances the gait function by reducing the muscle effort and metabolism while supporting the hip joint. Furthermore, the activities of the knee and ankle muscles is reduced, as is the activity of the hip flexors and extensors, suggesting that wearable hip exoskeletons can improve trunk stabilization during walking, particularly in older adults.3
Studies on the resistance torque of wearable hip exo-skeletons have shown that walking with the resistance provided by them offers both aerobic and resistance exercise benefits, significantly increasing trunk and lower limb strength compared to walking without the device.6 Aurich et al. analyzed electromyography (EMG) patterns in children with motor impairments walking on a treadmill using a wearable hip exoskeleton, and reported that the device increased quadriceps muscle activity.7 Studies involving healthy individuals walking with a wearable hip exoskeleton have also demonstrated either similar or higher EMG activity in the leg muscles compared with normal walking.8
Such EMG data can be used for the development and evaluation of wearable hip exoskeletons and can contribute to the understanding of physiological motor processes when using these devices.9 During walking, the trunk muscles play a crucial role in generating and coordinating movement between the trunk and pelvis, with abdominal muscles such as the external oblique (EO) and internal oblique (IO) stabilizing the spine and sacroiliac joints.10 Although wearable hip exoskeletons have been employed to improve gait function in patients with neurological disorders,11–12 the extent to which trunk muscles are activated during gait with a wearable hip exoskeleton remains unclear,13 and few studies have investigated muscle activation related to wearable hip exoskeleton use.
Therefore, this study aimed to investigate the effects of different modes of a wearable hip exoskeleton on muscle activation during walking in healthy adults by comparing muscle activation during normal-, assisted-, and resistance-mode walking. The hypothesis of this study is that muscle activation in the abdomen and lower limbs will increase during walking in resistance mode compared to normal walking and assisted mode walking.
METHODS
Participants were recruited from Baekseok University in Cheonan, Chungnam Province through online advertisements. The inclusion criteria were healthy individuals with no recent history of musculoskeletal or neurological disorders, and no surgical history. Exclusion criteria included weight exceeding 100 kg (treadmill weight limit) or BMI> 25 kg/m2. A total of 29 participants were recruited, and their general characteristics are presented in Table 1. While the sample size calculated through a power analysis using G*Power software was 18 participants, a total of 29 participants were included in this study. The larger sample size was implemented to enhance the reliability of the findings and increase statistical power for detecting differences in muscle activation across the different walking modes. The power analysis was based on an alpha level of 0.05, a power of 0.80, and an effect size of 0.60. This study was approved by the Institutional Review Board of Baekseok University (BUIRB-202310-HR-018). All participants provided written informed consent before participation.
Mean±SD | |
---|---|
Age (years) | 22.66±1.65a |
Height (cm) | 170.62±9.92 |
Weight (kg) | 65.34±12.48 |
BMI (kg/m2) | 22.22±2.03 |
Age, height, and weight were measured to assess the general characteristics of the participants, and BMI was calculated. The Bot Fit was applied in three different modes: general walking (GW), robotic-resistant walking (RRW), and robotic-assisted walking (RAW). In the GW mode, participants wore the Bot Fit without power assistance. For RRW, participants wore Bot fit with the resistance set to level 5. In the RAW mode, Bot Fit was set to provide assistance at level 5. To minimize order effects, the sequence of the modes was randomized. The participants walked on a treadmill at a constant speed of 4 km/h, representing the average walking speed across all three modes. Each mode was performed for 2 minutes and repeated three times, resulting in a total of 6 minutes per mode.14,15 A 3-minute rest period was provided between sessions to prevent fatigue. Muscle activation data were recorded during a 20-second interval in the middle of each walking session (1 min and 30 s into the session) to capture consistent activation levels, as established in previous study.16 The treadmill used in this study was the Xiaomi WalkingPad A1 (Xiaomi, Beijing, China).
The wearable hip exoskeleton used in this study was Bot Fit (Samsung Electronics Co., Ltd., Suwon, Korea), a device designed to provide assistance or resistance to hip flexion and extension during gait. Weighing 2.9 kg, Bot Fit consists of a pair of actuators, a hip brace, thigh frames, and fabric belts. It operates using a delayed output feedback control algorithm, which generates assistive or resistive torque based on the user’s hip joint angle and angular velocity.6 Bot Fit defines the hip joint angle to be 0° when the user is standing upright with the device on and is equipped with mechanical stoppers set to a maximum of 110° for flexion and 45° for extension, ensuring the joint remains within this safe range even in the event of device malfunction. Under normal conditions, the DOFC controller limits flexion to 100° and extension to 40°, with a working torque range of 0–12 Nm (or –12 to 0 Nm for resistance torque) and a maximum torque limited to 12 Nm+15%. Additionally, Bot Fit includes safety features such as a torque-off function that activates if an obstruction, like clothing, is detected. The device offers five levels of intensity for both assistance and resistance modes, and it can stop assistive or resistive forces if the user halts movement, resuming once the physical therapist resets the device and the user resumes walking.
The surface electromyography system Delsys Trigno (Trigno, Delsys, Natick, MA, USA) was used to measure and evaluate skeletal muscle contraction capacity and physiologically analyze muscle activity potentials. The data collected through the main device and attached sensors were processed using EMGworks Acquisition software (Delsys Inc., Natick, USA). The activities of the EO, IO, erector spinae at the level of L2 (ES), gluteus maximus (GM), rectus femoris (RF), and hamstring (HAM) were measured, with sensors attached to the right side. Following the SENIAM guidelines, the skin at the electrode attachment sites was shaved and cleaned with alcohol wipes before placing the electrodes.17 EMG signals were inspected to remove any non-physiological artifacts. The EMG data were bandpass filtered with a 4th-order zero-lag Butter-worth filter from 20-450 Hz and then rectified. Next, the EMG data were low-pass filtered at 10 Hz to create a linear envelope. The sampling rate was set to 1,000 Hz, and the extracted data were rectified and processed using the root-mean-square method. The EMG signals extracted during each walking mode were clipped based on the average peak value of each muscle (0.95 s) and normalized to the root mean square value of the participants’ maximum voluntary isometric contraction (MVIC).
To measure the MVIC of the external oblique, the participants laid in a supine position and performed left trunk rotation, raising the right shoulder blade off the bed, while the examiner applied resistance from left to right on the participant’s right shoulder.16 In the internal oblique position, MVIC was measured during right trunk rotation. When the left shoulder blade was lifted off the bed, the examiner applied resistance from right to left on the participant’s left shoulder.18 MVIC of the erector spinae was measured during back extension. In the prone position, the participants extended their lower back while the examiner applied resistance at the mid-thoracic level.19 The MVIC of the gluteus maximus was measured during hip extension. In the prone position, the participants extended their hips while the examiner applied downward resistance.20 MVIC of the rectus femoris was measured during knee extension. While seated on the edge of the bed, the participants had a strap placed around their ankles, and the examiner instructed them to perform knee extension.21 Hamstring MVIC was measured during knee flexion. In the prone position, the participants flexed their knees at a 30° angle while the examiner applied resistance in the direction of knee extension.22
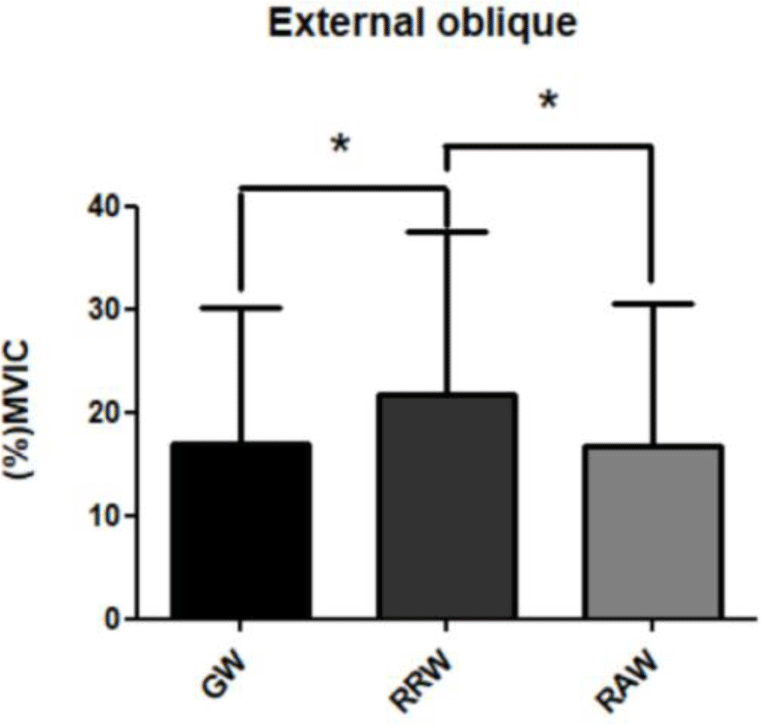
The data collected in this study were analyzed using SPSS ver. 18.0. The general characteristics of the partici-pants are presented as means and standard deviations using descriptive statistics. A Shapiro–Wilk test was conducted to test for normality, and because the data satisfied normality, parametric statistical methods were used for analysis. A repeated-measures analysis of variance was performed to compare the muscle activation across the different walking modes of the wearable hip exoskeleton, with a significance level set at p<0.05. To determine which modes showed statistical significance, Post hoc paired t-tests with Bonferroni correction were conducted to examine the statistical differences between each mode, with the significance level set at p<0.017.
RESULTS
The muscle activation values for the EO, IO, RF, and GM muscles were statistically significant across the different walking modes (Table 2). In the post hoc test, EO muscle activation was significantly higher in RRW than in GW and RAW (p<0.017). GM activation was also significantly higher in RRW than in GW and RAW (p<0.017). However, there were no statistically significant differences in muscle activation for the ES, RF, IO and HAM across the modes (p>0.017).
DISCUSSION
This study aimed to investigate the effects of wearable hip exoskeletons on the trunk and lower limb muscle activation in young adults during treadmill walking. The results revealed statistically significant differences in the activation of the EO muscles among the trunk muscles, with the highest activation observed in RRW. The resistance provided by the Bot Fit wearable hip exoskeleton is often described as being similar to walking in water, and this mode is sometimes referred to as the “aqua mode.” According to previous studies, walking in waist-deep water increases the load on the legs, requiring greater trunk muscle strength,23,24 which may explain the higher muscle activation observed in the EO during RRW. The resistance mode of the wearable hip exoskeleton and walking in water share some similarities, but they are not identical. Both conditions increase the load on the lower limbs and require greater muscle activation to maintain stability and propulsion. In the case of the Bot Fit wearable hip exoskeleton, the resistance applied during RRW is designed to simulate the effort needed to walk against an external force, which may mimic some aspects of the resistance experienced in water.
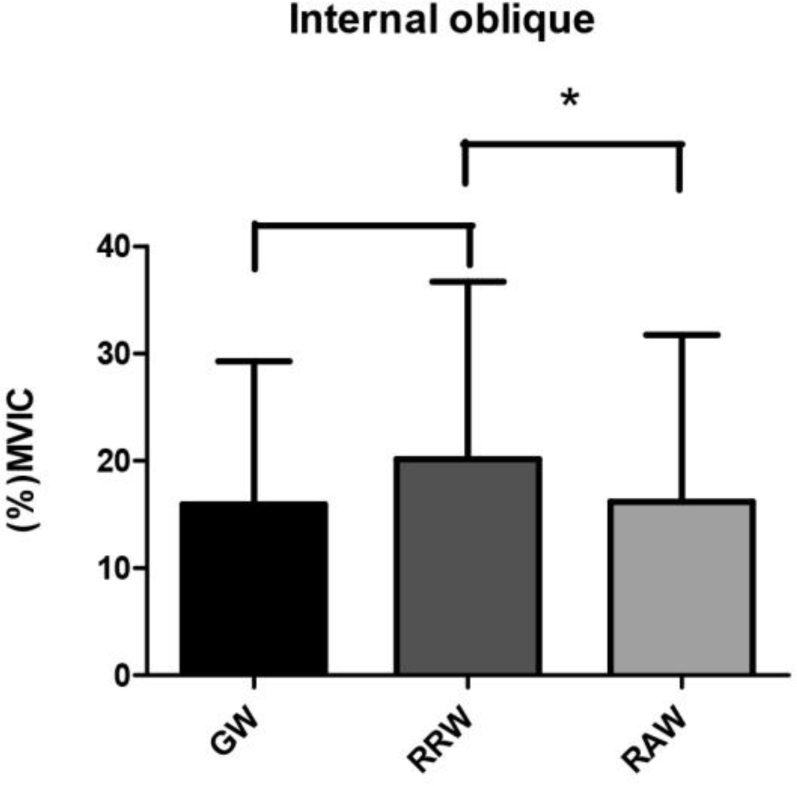
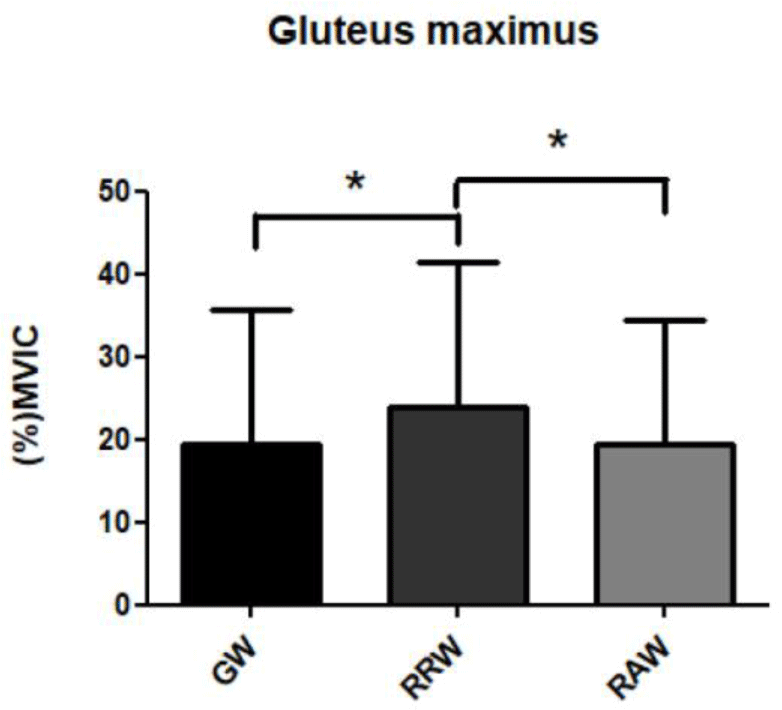
However, there are key differences between the two. Walking in water involves buoyancy, which reduces the impact on joints and alters the biomechanics of movement compared to land-based resistance provided by the exoskeleton. Additionally, the fluid dynamics of water introduce varying resistance depending on the speed and depth of the water, while the resistance from the exoskeleton is more controlled and consistent. Thus, while the analogy to “aqua mode” highlights the increased effort required during RRW, it is essential to recognize that the biomechanics and physiological responses may differ between the two modes.
GM activation was also the highest in RRW. The GM shows moderate activity during horizontal walking at a constant speed but becomes more active when propulsion is needed, helping the trunk move forward when the foot strikes the ground.25 Previous studies have emphasized the essential role of the GM in maintaining trunk stability and posture,26–28 and it appears that the GM is activated to counteract the resistance of the wearable hip exoskeleton and to maintain trunk posture, similar to the EO and IO. Additionally, previous studies have reported that GM st-rength is needed to accelerate during underwater walking,29 suggesting that the wearable hip exoskeleton, which simulates walking with a resistance similar to that of water, may have induced increased activation in the GM.
Although the RF showed statistically significant changes in muscle activation across modes, the differences were not significant in the mode comparisons. Previous studies have shown that the RF is active during the stance and early swing phases of the gait cycle, and its activation may vary significantly among individuals.30 In this study, individual differences in the timing, duration, and amplitude of RF activation likely contributed to the lack of significant differences between modes. Furthermore, Piazza et al. reported that RF activation is relatively low during slow walking,31 suggesting that the resistance and assistance provided in this study may have affected each participant’s walking pattern differently, leading to non-significant differences across the three modes.
The ES did not show statistically significant differences in activation across modes. Previous studies have reported that ES activation is observed when the foot strikes the ground during walking, but decreases during other phases.13 White et al. suggested that ES contraction prevents forward trunk rotation when the foot makes contact with the ground.32 Given the unclear role of the ES in walking and the fact that the wearable hip exoskeleton applied resistance and assistance to hip flexion, it appears that the wearable hip exoskeleton did not significantly influence ES activation.
This study has several limitations. First, it is challenging to generalize the findings as the study was conducted with healthy adults. Second, this study included a relatively limited sample size, which may restrict the generalizability of the findings to broader populations. Third, as the experiment was conducted exclusively in a treadmill environment, future research is necessary to evaluate the effects of exoskeleton use in more varied and realistic walking conditions.
CONCLUSIONS
The results of this study showed that the RRW mode of the wearable hip exoskeleton significantly increased muscle activation in the EO, GM. This suggests that walking with a wearable hip exoskeleton in the RRW can strengthen the key muscles that are important for trunk stabilization. Moreover, the increased activation of these muscles can play an important role in improving balance and walking efficiency, indicating that wearable hip exoskeletons have the potential to enhance both muscle activation and trunk stability, beyond their role as simple walking aids.